Background radiation is the ionizing radiation emitted from a variety of natural and artificial radiation sources. Primary contributions come from,
* Sources in the Earth. These include sources in our food and water, which are incorporated in our body, and in building materials and other products that incorporate those radioactive sources;
* Sources from space, in the form of cosmic rays;
* Sources in the atmosphere. One significant contribution comes from the radon gas is released from the earth's crust and subsequently decays to radioactive atoms that become attached to airborne dust and particulates. Another contribution arises from the radioactive atoms produced in the bombardment of atoms in the upper atmosphere by high-energy cosmic rays.
Today, a small fraction of background radiation also comes from radioactive tools such as smoke detectors, from self-luminous dials and signs, from global radioactive contamination due to historical nuclear weapons testing, nuclear power station or nuclear fuel reprocessing accidents, and from normal operation of the nuclear power industry. Sometimes included in background radiation are routine medical procedures like X-ray imaging; this is purposeful diagnostic exposure which dwarfs all other human-caused background radiation in the population of the industrialized world.
The Big Bang theory predicts that the early universe was a very hot place and that as it expands, the gas within it cools. Thus the universe should be filled with radiation that is literally the remnant heat left over from the Big Bang, called the “cosmic microwave background radiation”, or CMB.
The existence of the CMB radiation was first predicted by George Gamow in 1948, and by Ralph Alpher and Robert Herman in 1950. It was first observed inadvertently in 1965 by Arno Penzias and Robert Wilson at the Bell Telephone Laboratories in Murray Hill, New Jersey. The radiation was acting as a source of excess noise in a radio receiver they were building. Coincidentally, researchers at nearby Princeton University, led by Robert Dicke and including Dave Wilkinson of the WMAP science team, were devising an experiment to find the CMB. When they heard about the Bell Labs result they immediately realized that the CMB had been found. The result was a pair of papers in the Physical Review: one by Penzias and Wilson detailing the observations, and one by Dicke, Peebles, Roll, and Wilkinson giving the cosmological interpretation. Penzias and Wilson shared the 1978 Nobel prize in physics for their discovery. Today, the CMB radiation is very cold, only 2.725° above absolute zero, thus this radiation shines primarily in the microwave portion of the electromagnetic spectrum, and is invisible to the naked eye. However, it fills the universe and can be detected everywhere we look. In fact, if we could see microwaves, the entire sky would glow with a brightness that was astonishingly uniform in every direction. The picture at left shows a false color depiction of the temperature (brightness) of the CMB over the full sky (projected onto an oval, similar to a map of the Earth). The temperature is uniform to better than one part in a thousand! This uniformity is one compelling reason to interpret the radiation as remnant heat from the Big Bang; it would be very difficult to imagine a local source of radiation that was this uniform. In fact, many scientists have tried to devise alternative explanations for the source of this radiation but none have succeeded.
In below image, red denotes hotter fluctuations and blue and black denote cooler fluctuations around the average. These fluctuations are extremely small, representing deviations from the average of only about 1/100,000 of the average temperature of the observed background radiation.
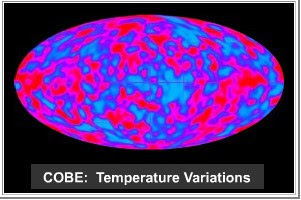
Why study the Cosmic Microwave Background?
Since light travels at a finite speed, astronomers observing distant objects are looking into the past. Most of the stars that are visible to the naked eye in the night sky are 10 to 100 light years away. Thus, we see them as they were 10 to 100 years ago. We observe Andromeda, the nearest big galaxy, as it was about 2.5 million years ago. Astronomers observing distant galaxies with the Hubble Space Telescope can see them as they were only a few billion years after the Big Bang. (Most cosmologists believe that the universe is between 12 and 14 billion years old.)
The CMB radiation was emitted only a few hundred thousand years after the Big Bang, long before stars or galaxies ever existed. Thus, by studying the detailed physical properties of the radiation, we can learn about conditions in the universe on very large scales, since the radiation we see today has traveled over such a large distance, and at very early times.
The Origin of the Cosmic Microwave Background
One of the profound observations of the 20th century is that the universe is expanding. This expansion implies the universe was smaller, denser and hotter in the distant past. When the visible universe was half its present size, the density of matter was eight times higher and the cosmic microwave background was twice as hot. When the visible universe was one hundredth of its present size, the cosmic microwave background was a hundred times hotter (273 degrees above absolute zero or 32 degrees Fahrenheit, the temperature at which water freezes to form ice on the Earth's surface). In addition to this cosmic microwave background radiation, the early universe was filled with hot hydrogen gas with a density of about 1000 atoms per cubic centimeter. When the visible universe was only one hundred millionth its present size, its temperature was 273 million degrees above absolute zero and the density of matter was comparable to the density of air at the Earth's surface. At these high temperatures, the hydrogen was completely ionized into free protons and electrons. Since the universe was so very hot through most of its early history, there were no atoms in the early universe, only free electrons and nuclei. (Nuclei are made of neutrons and protons). The cosmic microwave background photons easily scatter off of electrons. Thus, photons wandered through the early universe, just as optical light wanders through a dense fog. This process of multiple scattering produces what is called a “thermal” or “blackbody” spectrum of photons. According to the Big Bang theory, the frequency spectrum of the CMB should have this blackbody form. This was indeed measured with tremendous accuracy by the FIRAS experiment on NASA's COBE satellite.
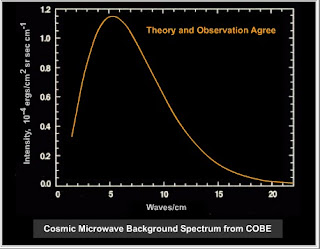
This figure shows the prediction of the Big Bang theory for the energy spectrum of the cosmic microwave background radiation compared to the observed energy spectrum. The FIRAS experiment measured the spectrum at 34 equally spaced points along the blackbody curve. The error bars on the data points are so small that they can not be seen under the predicted curve in the figure! There is no alternative theory yet proposed that predicts this energy spectrum. The accurate measurement of its shape was another important test of the Big Bang
“Wall of Light - Surface of Last Scattering”
Eventually, the universe cooled sufficiently that protons and electrons could combine to form neutral hydrogen. This was thought to occur roughly 400,000 years after the Big Bang when the universe was about one eleven hundredth its present size. Cosmic microwave background photons interact very weakly with neutral hydrogen.
he behavior of CMB photons moving through the early universe is analogous to the propagation of optical light through the Earth's atmosphere. Water droplets in a cloud are very effective at scattering light, while optical light moves freely through clear air. Thus, on a cloudy day, we can look through the air out towards the clouds, but can not see through the opaque clouds. Cosmologists studying the cosmic microwave background radiation can look through much of the universe back to when it was opaque: a view back to 400,000 years after the Big Bang. This “wall of light“ is called the surface of last scattering since it was the last time most of the CMB photons directly scattered off of matter. When we make maps of the temperature of the CMB, we are mapping this surface of last scattering.
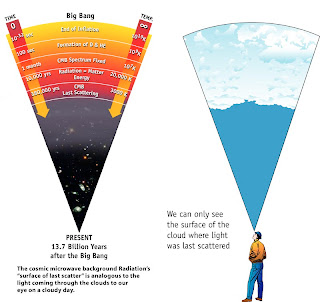
Problems with the Uniformity
The highly isotropic nature of the cosmic background radiation indicates that the early stages of the Universe were almost completely uniform. This raises two problems for the big bang theory.
First, when we look at the microwave background coming from widely separated parts of the sky it can be shown that these regions are too separated to have been able to communicate with each other even with signals travelling at light velocity. Thus, how did they know to have almost exactly the same temperature? This general problem is called the horizon problem.
Second, the present Universe is homogenous and isotropic, but only on very large scales. For scales the size of superclusters and smaller the luminous matter in the universe is quite lumpy, as illustrated in the following figure.
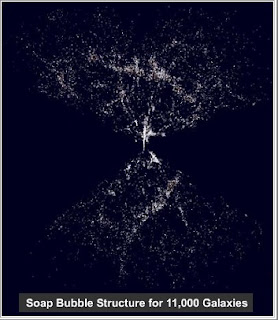
Data from the survey of galaxies. The voids and "walls" that form the large-scale structure are mapped here by 11,000 galaxies. Our galaxy, the Milky Way, is at the center. The outer radius is at a distance of approximately 450 million light-years. Obscuration by the plane of the Milky Way is responsible for the missing pie-shaped sectors to the right and left. Click on the image to get a larger version. (Smithsonian Astrophysical Observatory, 1993. Northern data (top)--Margaret Geller and John Huchra, Southern data (bottom)--Luiz da Costa et al. Quoted in Cosmology, a Research Briefing, National Academy of Sciences
What's "Cosmic" about it?
We refer to it as "cosmic" because the only known source of this radiation is the early universe. It can now be firmly concluded that the CMB is the cooled remnant of the hot Big Bang itself.
Why "Microwave"?
Light comes in a range of wavelengths, from the shortest wavelength gamma-rays to the longest wavelength radio waves, with common-or-garden visible light in the middle. All of these signals are manifestations of the same underlying physical phenomenon, travelling packets of oscillating electric and magnetic fields, called electro-magnetic radiation. All of the forms of electromagnetic radiation travel at the same speed, the speed of light, which is 300,000 km/s. E-m radiation of different wavelengths will interact with matter in different ways. For example, radio waves are picked up by a radio receiver, your eye detects visible light, infra-red radiation warms your skin, x-rays penetrate your body, gamma-rays can give you radiation damage.
Microwaves are the name given to radiation between the infra-red and radio region, with wavelengths typically in the 1mm to 10cm range. Some specific wavelengths of microwaves can be used to excite the molecules in foodstuff, so that you can use them to cook. It turns out that if you had a sensitive microwave telescope in your house you would detect a faint signal leaking out of your microwave oven, and from various other man-made sources, but also a faint signal coming from all directions that you pointed. This is the Cosmic Microwave Background.
Why is it called a "Background"?
We refer to this radiation as a background because we see it no matter where we look. It clearly doesn't come from any nearby objects, such as stars or clouds within our Galaxy, or even from external galaxies. It is clearly a distant, "background" source of radiation. You can think of the whole Universe as being filled with this background of microwave photons.
How do I pronounce "anisotropy"?
If you've never come across this word before, then (obviously) it's new to you, and so even professional cosmologists sometimes pronounce it wrongly. This then is a good question, but hard to answer in plain text! Basically, the stress is on the third syllable, and the common mistake is to stress the fourth. The confusion presumably arises from knowing how to pronounce "anisotropic", and then thinking that you just pronounce it the same way, but without the final consonant.
Why does the CMB support the Big Bang picture?
The basic point is that the spectrum of the CMB is remarkably close to the theoretical spectrum of what is known as a "blackbody", which means an object in "thermal equilibrium". Thermal equilibrium means that the object has had long enough to settle down to its natural state. Your average piece of hot, glowing coal, for example, is not in very good thermal equlibrium, and a "blackbody" spectrum is only a crude approximation for the spectrum of glowing embers. But it turns out that the early Universe was in very good thermal equilibrium (basically because the timescale for settling down was very much shorter than the expansion timescale for the Universe). And hence radiation from those very early times should have a spectrum very close to that of a blackbody.
The observed CMB spectrum is in fact better than the best blackbody spectrum we can make in a laboratory! So it is very hard to imagine that the CMB comes from emission from any normal "stuff" (since if you try to make "stuff" at some temperature, it will tend to either emit or absorb preferentially at particular wavelengths). The only plausible explanation for having this uniform radiation, with such a precise blackbody spectrum, is for it to come from the whole Universe at a time when it was much hotter and denser than it is now. Hence the CMB spectrum is essentially incontrovertible evidence that the Universe experienced a "hot Big Bang" stage (that's not to say that we understand the initial instant, just that we know the Universe used to be very hot and dense and has been expanding ever since).
In full, the three cornerstones of the Big Bang model are: (1) the blackbody nature of the CMB spectrum; (2) redshifting of distant galaxies (indicating approximately uniform expansion); and (3) the observed abundances of light elements (in particular helium and heavy hydrogen), indicating that they were "cooked" throughout the Universe at early times. Because of these three basic facts, all of which have strengthened over the decades since they were discovered, and several supporting pieces of evidence found in the last deacade or two, the Big Bang model has become the standard picture for the evolution of our Universe.
Can I see the CMB for myself?
In fact you can! If you tune your TV set between channels, a few percent of the "snow" that you see on your screen is noise caused by the background of microwaves.
How come we can tell what motion we have with respect to the CMB?
Doesn't this mean there's an absolute frame of reference?
The theory of special relativity is based on the principle that there are no preferred reference frames. In other words, the whole of Einstein's theory rests on the assumption that physics works the same irrespective of what speed and direction you have. So the fact that there is a frame of reference in which there is no motion through the CMB would appear to violate special relativity!
However, the crucial assumption of Einstein's theory is not that there are no special frames, but that there are no special frames where the laws of physics are different. There clearly is a frame where the CMB is at rest, and so this is, in some sense, the rest frame of the Universe. But for doing any physics experiment, any other frame is as good as this one. So the only difference is that in the CMB rest frame you measure no velocity with respect to the CMB photons, but that does not imply any fundamental difference in the laws of physics.
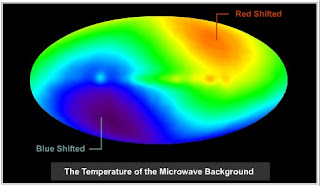
What sort of telescope is used to observe the CMB?
Like light at any other wavelength the general system is a dish to collect and focus the radiation, a way of feeding the radiation to the instruments, and then the instruments themselves which are used to detect and record the signals. For microwaves the dish, or set of dishes, is made of a material (metal) which reflects microwaves. The focussed radiation is transported to the receivers by means of "wave-guides", which are pipes specially tuned to transmit microwave signals.
Resources:
http://map.gsfc.nasa.gov/m_uni/uni_101bbtest3.html
http://csep10.phys.utk.edu/astr162/lect/cosmology/cbr.html
http://en.wikipedia.org/wiki/Background_radiation
http://www.astro.ubc.ca/people/scott/faq_basic.html